Abstract
TP53 is a tumor suppressor gene frequently mutated in human cancers and is generally associated with poor outcomes. TP53 mutations are found in approximately 5% to 10% of patients with de novo acute myeloid leukemia (AML), more frequently observed in elderly patients and those with therapy-related AML. Despite recent advances in molecular profiling and the emergence of targeted therapies, TP53-mutated AML remains a challenge to treat. Current treatment strategies, including conventional chemotherapy, hypomethylating agents, and venetoclax-based therapies, have shown limited efficacy in TP53-mutated AML, with low response rates and poor overall survival. Allogeneic hematopoietic stem cell transplantation is a potentially curative option; however, its efficacy in TP53-mutated AML depends on comorbid conditions and disease status at transplantation. Novel therapeutic modalities, including immune-based therapies, did show promise in early-phase studies but did not translate into effective therapies in randomized controlled trials. This review provides a comprehensive overview of TP53 mutations in AML, outcomes based on allelic burden, clinical implications, and therapeutic challenges.
Similar content being viewed by others
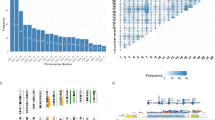
Introduction
The TP53 gene, also known as the guardian of the genome, is a tumor suppressor gene located on chromosome 17p13.1 and is the most frequently mutated gene in human cancers [1]. It encodes for 393 amino-acid phosphoprotein (p53), a transcription factor critical for cell cycle regulation, DNA repair, apoptosis, and metabolic pathways [2]. TP53 mutations are more common in solid cancers, found in 10% to 15% of acute myeloid leukemia (AML) cases [3]. TP53 mutations are found in 5% to 10% of de novo AML, more frequently observed in patients with therapy-related AML (t-AML), adult pure erythroid leukemia, and cases with complex karyotypes [4].
Integration of molecular genetics analysis through techniques like next-generation sequencing has changed the classification and prognostication of cancers [5]. Given its unique features and outcomes, TP53-mutated AML is now classified as a separate disease entity from other subtypes of AML per the European LeukemiaNet (ELN) and International Consensus Classification (ICC) [6, 7]. TP53-mutated AML is a poor prognostic disease with suboptimal response to conventional therapies, where allogeneic hematopoietic stem cell transplantation can secure long-term remission in only 10–15% of eligible patients [8, 9]. Poor prognosis in the current era of novel therapy is rapidly being recognized, and specific strategies to treat TP53-mutated AML are being pursued. In this review, we discuss challenges in managing TP53-mutated AML and potential strategies to improve long-term outcomes.
TP53 and Oncogenesis
TP53 is normally inactive, and posttranslational modifications such as continuous ubiquitylation cause subsequent degradation, which allows the unstressed cell to maintain a low level of WT-p53 [10]. When activated under cellular stress, p53 acts as a transcriptional activator of more than 150 target genes, controlling cellular mechanisms, including cell cycle arrest, DNA damage repair, senescence, and apoptosis (Fig. 1). Cell cycle arrest and apoptosis are mediated by p53 binding to genes such as p21/WAF and Bcl-XL [11].
TP53 is pivotal in controlling self-renewal and proliferation of normal hematopoietic stem cells. Disruption in TP53 results in the overproduction of pluripotent stem cells, leading to leukemogenesis [12]. TP53 mutations produce altered p53, which is nonfunctional and can also exert a dominant-negative effect on the residual WT-p53. A considerable proportion (70–80%) of these mutations are missense substitutions encoded by exons 5 through 8, causing amino-acid changes, and the rest are truncating alterations [13]. Three main functional regions of TP53 are the amino-terminal region, the centrally located DNA-binding domain, and the oligomerization domain. Mutations in the DNA-binding domain lead to the functional inactivation of p53 and the inability to transactivate target genes, causing the loss-of-function variant [12]. Mutations in the oligomerization domain culminate in the loss of both tetramerization and tumor suppression function [12]. Surprisingly, amino-terminal region mutations activate the p47 protein, which makes cells more sensitive to therapies and thus improves survival [14]. Often, mutant p53 confers gain-of-function properties that enhance metastasis and increase drug resistance and survival of tumor cells. This is achieved by alterations and inhibition of tumor suppressor genes and transcriptional factors, such as p63 and p73, essential for cell cycle arrest [15]. Overexpression of p53-negative regulators MDM2 and MDM4 are other mutational mechanisms [16].
TP53 mutation allelic burden and outcome
TP53 allelic state, co-occurring somatic mutations, and position within the clonal hierarchy depict the genetic heterogeneity in TP53-mutated myeloid malignancies [17]. In monoallelic TP53 mutations, the WT allele is preserved, while in biallelic mutations, the second TP53 allele is inactivated by mechanisms such as point mutation, loss of heterozygosity (LOH) or copy neutral LOH (cnLOH). Biallelic TP53 mutations are associated with complex karyotype, higher blast count, and higher leukemic transformation [17, 18]. The ICC defines TP53-mutated myeloid neoplasm into three distinct categories: multi-hit myelodysplastic syndrome (MDS; bone marrow and blood myeloid blasts 0%-9%), MDS/AML (bone marrow and blood myeloblasts 10–19%), and AML (bone marrow or blood myeloblast ≥20%) irrespective of allelic loss with overall dismal prognosis [7]. Multi-hit status is defined as the presence of ≥2 distinct TP53 mutations (variant allele frequency [VAF] of each mutation ≥10%) or a single TP53 mutation associated with 1) deletion involving the TP53 locus at 17p13.1 evident on cytogenetic analysis; 2) a VAF ≥ 50%; or 3) cnLOH at the 17p TP53 locus. In the absence of comprehensive copy number analysis, the presence of a complex karyotype is considered a multi-hit equivalent. While a single hit is defined as one TP53 mutation with VAF ≥ 10% to <50% without LOH or cnLOH at 17p13.1 locus.
There is a consensus on the poorer prognostic significance of multi-hit vs. single-hit TP53 mutation in low-risk MDS [17]. However, the differences in clinical outcomes between multi-hit and single-hit TP53 mutation in high-risk MDS and AML are less clear [18, 19] and is actively debated. Furthermore, there is a lack of consensus about terminology, the definition of biallelic loss, and prognostic significance between the recently published World Health Organization (WHO) classification 5th edition (WHO-5) and ICC. WHO-5 defines a single entity of biallelic TP53 MDS regardless of blast percentage (0-19%) with poor prognosis. Monoallelic MDS is considered to have a good prognosis, similar to TP53 wild-type MDS, and is excluded from the TP53-mutated MDS [20]. This classification is predominantly driven by landmark analysis by Bernard et al. [17] of 3,324 patients with MDS for TP53 mutations and allelic imbalances. The monoallelic loss was detected in one-third, and bi-allelic alterations in two-thirds of those with TP53-mutated MDS. They demonstrated TP53 bi-allelic loss as a predictor of mortality and leukemic transformation independent of the Revised International Prognostic Scoring System (IPSS-R). Monoallelic losses were found to have outcomes and therapeutic responses similar to TP53-WT [17]. Grob et al. [19] studied 2,200 patients with either TP53-mutated AML or MDS with excess blasts (MDS-EB) treated with intensive chemotherapy using next-generation sequencing and found a 2-year overall survival (OS) of 12.8% in TP53-mutated AML. Contrary to the Bernard et al. study, this study reported no difference in OS between AML and MDS-EB, irrespective of allelic status. They proposed considering TP53-mutated MDS-EB and AML a single entity due to molecular homogeneity, distinct clinicopathologic characteristics, and inferior outcomes regardless of blast percentage at diagnosis.
TP53 in MN in the context of blast percentage
Regardless of blast count percentage, TP53-mutated myeloid neoplasms (MN) have aggressive clinical behavior with a dismal prognosis [7]. The ICC and updated ELN guidelines considered TP53-mutated MN an independent group in the adverse prognostic risk category [6, 7]. Based on the presence and specific characteristics of TP53 mutations, a novel MDS subcategory was added to the classification of myeloid malignancies by both the ICC and WHO [20]. In the 2017 (revised 4th edition) WHO classification, a blast count of 20% or greater in marrow and/or blood was needed to diagnose most cases of AML [21]. Changes have been made in the classification of MN based on blast percentage in the 2022 classifications from both the ICC and WHO [7, 20]. In the WHO 5th edition classification, AML is divided into 2 major groups: AML with defining genetic abnormalities and AML defined by differentiation (previously AML, NOS). The blast cutoff of 20% has been removed from AML with defining genetic abnormalities except for AML with BCR::ABL1 or AML with CEBPA mutation with the former to avoid overlap with chronic myeloid leukemia. This is based on evidence suggesting that clinical outcomes of AML with a low blast percentage are comparable to AML with a high blast percentage when certain genetic abnormalities are present [20].
In contrast, the 2022 ICC classification now requires a blast cutoff of 10% for AML with recurrent genetic abnormalities, except for AML with BCR::ABL1, for which the 20% blast cutoff persists. A new category of MDS/AML has been introduced for adult cases with 10% to 19% blasts, eliminating the revised 4th edition WHO category of MDS-EB2 in adults [7]. Genetics, rather than arbitrary blast count cutoff, may drive management decisions in this subgroup [22]. The recommended blast count threshold for MDS remains below 10% in both classifications [7, 20]. Under the 2022 ICC guidelines, MDS, MDS/AML, and AML (all with mutated TP53) are separate entities defined by blast percentages of less than 10%, 10% to 19%, and 20% or greater, respectively, with biallelic loss required for the less than 10% group. However, these diseases show overall aggressive behavior and warrant a novel treatment strategy [7, 19] Hopefully, newer classifications, relying more on genomic-driven prognosis rather than mere blast count, will facilitate clinical trial design and stimulate research, culminating in better treatment strategies. Moreover, newer classifications of MDS/AML will create opportunities for patients with high-risk MDS to be enrolled in AML trials, especially those with TP53 mutations.
TP53 and therapy-related AML
In the ICC, prior cytotoxic therapy is considered a diagnostic qualifier rather than a feature definition of a disease entity. Features of t-AML that distinguish it from de novo AML include enrichment of TP53 mutations, complex cytogenetics, abnormalities of chromosomes 5 and 7, and suboptimal response to conventional chemotherapy [23] but these findings overlap with AML arising from prior MDS. The likelihood of TP53 mutations in t-AML is 25% to 40% compared to 5% to 10% in de novo AML [23, 24]. It is unclear whether prior cytotoxic exposure is responsible for leukemogenesis and selective enrichment of TP53 mutations. Wong et al. [25] conducted genomic sequencing in a cohort of 22 patients with t-AML, revealing that the total number of somatic single-nucleotide variants and proportion of cytotoxic therapy-related transversion were comparable in t-AML and de novo AML. Several patients with t-AML/t-MDS had TP53 mutation at a lower frequency ( < 1.0%) before the development of t-AML/t-MDS [25]. Their observation suggests that chemotherapy does not induce TP53 mutations rather smaller TP53 mutation clones preferentially expand after chemotherapy and result in MN [25]. Since TP53 activation is essential for chemotherapy-induced cellular apoptosis, enriched levels of mutated TP53 in t-AML could explain poor response to conventional chemotherapy [26].
In t-MDS, TP53 mutations are associated with a high risk of progression to AML. This subset of t-MDS is highly chemo-refractory and portends dismal outcomes with a 5-year OS of less than 2% [27, 28]. Recently, Hiwase et al. [4] evaluated the prognostic impact of TP53 mutation allelic status on therapy-related MN outcomes. The study demonstrated no difference in clinical features, genomic stability, and pattern of co-mutations between single- and multi-hit TP53 MN. Disease latency, blood counts, blast percentage, cytogenetic aberrations, and OS were similar between single- and multi-hit t-AML. TP53 mutation with VAF ≥ 10% was validated as a predictor of poor outcome in this study [4]. Collectively, these studies confirm poor survival of TP53-mutated MN regardless of the context (de novo vs. therapy-related) and highlight the unique biology of therapy-related TP53-mutated MN [29].
Conventional therapies for TP53-mutated AML
Historically, the combination of cytarabine with anthracyclines or a high-dose cytarabine-based combination was commonly used as a frontline regimen among patients eligible for intensive chemotherapy [30]. For ineligible patients (i.e., those with advanced age and multiple comorbid conditions), hypomethylating agents (HMAs) alone or in combination with investigational agents were the mainstay of treatment [31]. With few exceptions, the same algorithm was used to manage TP53-mutated AML, and it continued to show dismal outcomes due to inherent resistance to conventional therapies [32].
Cytotoxic induction chemotherapy for TP53-mutated AML tends to have a poor response (28–42%) and short OS of 5-9 months [3, 28, 33]. However, the presence of TP53 mutation is not an indicator to forego treatment, as disease progression is far worse without chemotherapy [34]. Historically, an anthracycline-based 7 + 3 regimen (7 days of cytarabine and 3 days of daunorubicin) was used as frontline therapy in eligible patients with a complete remission (CR) rate between 20% and 40% [35]. Liposomal daunorubicin and cytarabine (CPX-351) is a dual-drug encapsulated formulation that preferentially delivers a synergistic 5:1 drug ratio into leukemia cells, avoiding normal bone marrow cells [36]. CPX-351 has been approved by the Food and Drug Administration for secondary AML and t-AML induction and has demonstrated significantly prolonged median OS (9.56 months vs. 5.95 months) and improved CR rates (47.7% vs. 33.3%) compared a 7 + 3 regimen, with a comparable safety profile [37]. Subsequently, Goldberg et al. [37] studied CPX-351 in TP53-mutated AML and found lower CR/CR with incomplete count recovery (CRi) compared to WT TP53 AML (33% vs. 66%; P = 0.0353), suggesting liposomal formulation of anthracycline and cytarabine may not be as effective in this disease entity compared to secondary AML with WT TP53. Other studies have reported low CR/CRi with CPX-351 in TP53-mutated AML vs WT TP53 AML [38,39,40,41,42,43].
The second major group are HMAs, include azacitidine (AZA) and decitabine, which were the preferred frontline therapy in elderly patients not eligible for induction therapy before the venetoclax (VEN) era [44]. Studies have reported increased sensitivity of p53-deficient cells to HMA in preclinical settings [45]. Welch et al. [46] conducted a single-center study in 116 patients with TP53-mutated MDS/AML and reported favorable clinical response and robust (but incomplete) mutation clearance in AML and MDS with unfavorable risk cytogenetics and TP53 mutations or both when treated with 10 days of decitabine in a 28-day cycle. This cohort had similar OS compared to patients with AML with intermediate-risk cytogenetics profiles treated with 10 days of decitabine [46]. In contrast, Short et al. [47] reported comparable outcomes with 10-day vs. 5-day decitabine therapy among patients with TP53-mutated AML. While there is debate over the optimal length of decitabine therapy for TP53-mutated AML (10 days vs. 5 days), HMA-based therapy was the front runner for the management of elderly patients with AML with adverse-risk disease, including TP53 mutations, before VEN was approved in combination with HMAs [48].
Venetoclax-Based Therapies for TP53-Mutated AML
Venetoclax (VEN), a B-Cell lymphoma-2 protein inhibitor, has shown promising efficacy in combination with AZA in elderly patients with AML ineligible for intensive induction, demonstrating superior CR/CRi rates (66.4% vs. 28.3%) and a median OS of 14.7 months compared to 9.6 months with AZA alone [49]. However, the outcomes in TP53-mutated AML were dismal, and the hazard ratio did not show a survival benefit of VEN, when combined with AZA compared to AZA alone [49]. We and several other groups have reported improved response rates with the HMA + VEN combination in TP53-mutated AML; however, it did not translate into improved relapse-free or OS when compared to HMA alone, possibly due to the emergence of resistance to TP53 mutation resulting in early relapses and dismal survival [50,51,52].
A recently published meta-analysis by Daver et al. [35] on treatment-naïve TP53-mutated AML showcased improved CR with intensive chemotherapy (IC) and VEN + HMA compared to HMA alone (43% vs. 33% vs. 13%), but there was no significant OS difference across the treatment groups. Similarly, DiNardo et al. [53] reported CR/CRi of 47% in TP53-mutated AML with VEN + DEC/AZA, along with a median duration of response (DOR) of 5.6 months and median OS of 7.2 months. Data suggest modest improvement in CR/CRi compared to historically reported values as low as 18% [53]. Another retrospective analysis from the Mayo Clinic, that analyzed VEN + HMA in patients with treatment-naïve AML showed adaptive resistance to therapy and shorter OS in patients with TP53 mutation [54]. Pollyea et al. [55] reported improved composite remission rates with VEN + AZA but not DOR and OS compared to AZA alone in patients with treatment naïve AML harboring poor-risk cytogenetics and TP53 mutations. In a recent study by Gangat et al. [56] exploring VEN + HMA efficacy in newly diagnosed AML, multi-hit TP53 mutations were associated with lower CR/CRi compared to single-hit TP53 or WT mutations. The presence of TP53 mutation also conferred lower relapse-free survival (7.9 vs. 19.3 months) and poor OS (5.9 vs. 16.6 months). While VEN + HMA-based therapies have shown improved response rates compared to HMA alone, responses seem to be short-lived. Hence, we need better therapies with long-lasting response for this poor-risk disease.
Immune-based therapies for TP53-mutated AML
In recent years, immunotherapy has gained a spotlight in oncology. Immunotherapy agents rely on the innate ability of the immune system to detect and eliminate tumor cells. This treatment is being explored by investigators for the management of TP53-mutated AML [48]. Immune checkpoint inhibitors, bispecific and dual-antigen-receptor targeting antibodies, chimeric antigen receptor (CAR) T-cell therapy, and newer targets, such as T-cell immunoglobulin and mucin domain 3 (TIM-3) inhibitors and stimulators of interferon genes are some novel treatment modalities under this domain (Fig. 2) [57].
Checkpoint inhibitors function by blocking inhibitory co-receptors on T cells, including PD1, PD-L1, and cytotoxic T-lymphocyte antigen 4 inhibitors. These proteins are accessories to immune evasion by tumor cells, and the expression of these receptors and their ligands is augmented in myeloid malignancies, suggesting a possible resistance mechanism to conventional therapies [58, 59]. TP53-mutated AML is an immunologically distinct subset with high PD-1 expression compared to wild-type TP53. This mutation is linked to an immune-privileged phenotype characterized by increased PDL1, upregulation of MYC, and downregulation of miR-34a. Additionally, there is a reduction in cytotoxic T cells and helper T cells, along with an expansion of immunosuppressive regulatory T cells and myeloid-derived suppressor cells, contributing to immune evasion and poor clinical outcomes [60].
Checkpoint inhibitor combinations with HMA and intensive chemotherapy have been evaluated in clinical trials with some efficacy. In a recent phase 2 study, nivolumab was used in combination with AZA in patients with relapsed refractory (R/R) AML, including 16 patients with TP53 mutation [61]. The overall response rate (ORR) was 33%, CR/CRi was 22%, and the median OS was 6.3 months. A higher response rate was documented among patients who had not received HMA than those pretreated with HMA (ORR 5% vs 22%). Three of 16 patients with TP53-mutated AML showed a response (ORR 19%) [61]. Similarly, another phase II trial showed that pembrolizumab after high-dose cytarabine was safe in 37 patients with R/R AML (ORR 46%, CR 38%, median OS 11.1 months). Two of the 5 (40%) patients with TP53 mutation in this cohort achieved CR [62]. Then, in a phase II trial of upfront nivolumab with idarubicin and cytarabine in 44 treatment-naïve patients with high-risk MDS/AML, including 8 with TP53-mutated MDS, the CR/CRi rate was 78% [63]. Prospective, randomized studies are needed to assess if patients with TP53-mutated AML have better outcomes with checkpoint inhibitor combinations than with conventional chemotherapies alone.
Sabatolimab, a humanized monoclonal antibody targeting the human TIM-3, is a member of immunoregulatory receptor proteins [64]. The drug was evaluated in patients with high-risk MDS/AML in a phase Ib study, and durable responses were observed in patients with adverse-risk mutation, including TP53. Among patients with high-risk MDS, ORR was 71.4%, and median DOR was 21.5 months (95% CI, 6.7-not evaluable [NE]) [65]. Similarly, in patients with newly diagnosed AML with at least one adverse-risk mutation (TP53, RUNX1, or ASXL1), ORR was 53.8% and median DOR was 12.6 months (95% CI, 1.3-NE). The STIMULUS-AML-1 trial is ongoing to evaluate the safety and efficacy of sabatolimab in combination with VEN + AZA in patients with newly diagnosed AML not eligible for intensive chemotherapy [66].
CD-47 is an antiphagocytic expressed in cancer cells that interacts with SIRPα on macrophages and helps them escape phagocytosis. Magrolimab, an anti-CD47 antibody, blocks CD-47-SIRPα interaction on macrophages and enhances tumor cell death through phagocytosis [67]. It showed promising efficacy and tolerability in phase Ib trials. A report by Daver et al. [68] evaluated treatment with a magrolimab+AZA combination for 87 patients with treatment-naïve AML, including 72 patients with TP53-mutated AML (82.8%). Among the patients with TP53-mutated AML, CR was achieved in 31.9% (95% CI, 21.4–44.0), and CR/CRi was achieved in 40.3% (95% CI, 28.9–52.5). The median DOR was 7.7 months (95% CI, 4.7–9.7). Among 14 patients with TP53 mutations who achieved CR/CRi and were flow MRD-negative, the median OS was 14.5 months (95% CI, 12.1–21.7) vs 7.5 months (95% CI, 4.5–10.8) among patients who remained MRD-positive, regardless of response [68]. Subsequently, randomized studies were initiated to evaluate the outcomes of older/ineligible patients with TP53-mutated AML treated with magrolimab+AZA vs. investigator choice (ENHANCE-2) and AZA + VEN+magrolimab vs AZA + VEN (ENHANCE-3) [69]. However, magrolimab trials in AML recently have been discontinued due to futility based on planned analysis in comparison to standard-of-care therapy in TP53-mutated AML [70]. Nevertheless, ASPEN-02 and ASPEN-05 trials are investigating the role of another next-generation CD-47 blocker, evorpacept, in R/R AML [71, 72]. Prospective studies with clinical outcome among patients with TP53-mutated versus WT TP53 are highlighted in Table 1.
Bispecific antibodies, bispecific T-cell engagers, and dual affinity targeting antibodies have been under exploration for the management of TP53-mutated AML. Flotetuzumab, a CD123 and CD3-targeting dual affinity antibody that works by enhancing the formation of an immunologic synapse between cytotoxic T cells and AML cells independent of the major histocompatibility complex (MHC) pathway, has shown promising efficacy in R/R TP53-mutated AML (CR 47%, median OS 10.3 months) [73]. Another bispecific antibody against CD123 and CD3 (APVO436-5001) has shown efficacy and safety in an early-phase clinical trial [74].
CAR T cells are progressing as a highly efficacious therapy for B-cell malignancies, with a more manageable toxicity profile than allogeneic hematopoietic stem cell transplantation. Progress of CAR T cells in AML is stalled by off-target effects, myelosuppression, and poor CAR T-cell persistence [75]. Innovative strategies are being implemented to improve CAR T-cell efficacy in AML by targeting leukemia-specific antigens, gene editing, and using allogenic donor CAR T cells. CAR T cells against CD70 and CLL-1 antigen, which are less readily expressed on hematopoietic stem cells, are being actively explored for the management of R/R AML [76, 77]. While there is no significant data on CAR T cell therapy for TP53-mutated AML, it needs to be explored in future trials to improve the outcome of this poor prognostic disease. Table 2 lists the ongoing clinical trials for TP53-mutated MN.
Ongoing trials of novel molecular targets in TP53-mutated AML
Syk inhibitors
Splenic tyrosine kinase (Syk) is overexpressed in AML, and overactivity is associated with poor prognosis. Entospletinib is a splenic tyrosine kinase inhibitor that was evaluated in combination with decitabine for AML with TP53 mutation or complex cytogenetics in the Beat AML Master Trial [78]. The drug combination showed modest activity, and the study was discontinued due to futility [78].
ROS1 inhibitor
Several cancers, including AML, exhibit abnormal ROS proto-oncogene 1 receptor tyrosine kinase expression and are considered a potential target for anticancer therapies. A phase I trial is currently evaluating entrecitinib, a ROS proto-oncogene 1 receptor tyrosine kinase inhibitor, in combination with oral decitabine for the management of relapsed/refractory TP53-mutated AML [79].
Arsenic trioxide
A potent drug for treating acute promyelocytic leukemia, has shown promise as a treatment for TP53-mutated AML. In preclinical studies, arsenic trioxide has been shown to rescue mutated p53 by stabilizing the DNA-binding domain [80]. A phase II trial is being conducted to evaluate the efficacy of arsenic trioxide in combination with decitabine and cytarabine in TP53-mutated AML [81].
Statins
Similarly, statins have been shown to degrade misfolded p53 mutant proteins in preclinical studies and are being explored for the treatment of AML [82]. Studies have shown that TP53-mutated AML needs a mevalonate pathway for chemotherapy resistance, and targeting this pathway with statins in combination with chemotherapy may be beneficial [83]. Other novel therapies, with or without intensive chemotherapy or HMA, are under investigation for the management of TP53-mutated AML (Table 2).
Role of allogeneic hematopoietic cell transplantation in TP53-mutated AML
Allogeneic hematopoietic cell transplantation (allo-HCT) is a potential option to improve the long-term outcome of adverse-risk AML. However, the outcome of TP53-mutated AML with allo-HCT has been generally sub-optimal. Factors that can potentially impact outcome are co-occurring somatic mutation, complex cytogenetics, missense vs. truncating TP53 mutation, status of remission before allo-HCT, conditioning intensity, and post-transplant strategy [84]. Ciurea et al. [85] reported that patients with TP53-mutated MDS/AML do not uniformly show poor outcomes with allo-HSCT. They stratified patients based on the HCT comorbidity index (HCT-CI), Karnofsky performance status, and disease status at the time of transplant. Patients with 1 or more of the following risk factors had dismal survival: HCT-CI > 4, Karnofsky performance status ≤80%, and residual leukemia at the time of transplant [85].
The European Society for Blood and Marrow Transplantation (EBMT) evaluated the outcomes of patients with TP53-mutated AML receiving allo-HCT in CR1 [86]. Patients with concurrent complex cytogenetics or loss of 17p had poorer outcomes, with 2-year leukemia-free survival of 15%, while patients with no evidence of complex cytogenetics or 17p loss had a 2-year OS comparable to that of patients with preserved TP53 function [86]. Similarly, other studies suggested inferior outcomes with concurrent complex cytogenetics in TP53-mutated AML [19, 87]. Among a cohort of 202 patients with TP53-mutated AML, improved OS was observed with allo-HSCT (17.6 vs. 9.1 months; P = 0.006); OS benefit was more pronounced among patients with TP53 mutation VAF of 40% or less (32.2 vs. 9.5 months; P = .01) [88].
Missense mutation clustering in the DNA-binding domain is the most common variant in TP53-mutated AML. Whether clinical outcomes after allo-HSCT differ between missense and truncating variants is less well-established [89]. Lindsley et al. [90] analyzed the outcomes of 289 patients with TP53-mutated MDS who received allo-HCT and observed inferior outcomes among patients with truncating TP53 variants. The implications of the type of TP53 variant on the outcome of patients with AML receiving allo-HCT are not well established and need to be explored further.
In general, myeloablative conditioning is associated with better relapse-free survival compared to reduced-intensity conditioning in AML. However, it does not seem to hold in TP53-mutated AML [90]. In the EBMT registry study and our real-world analysis did not observe a significant impact of conditioning intensity on the outcome of patients with TP53-mutated AML receiving allo-HCT [9, 86]. In the contemporary era, a phase Ib study was conducted by adding VEN to fludarabine and busulfan (reduced intensity conditioning) for allo-HCT in patients with high-risk MDS/AML [91]. Among the 22 patients enrolled, 12 (55%) had TP53 mutation. The engraftment rate did not decrease, and the incidence of graft-versus-host disease did not increase. At 14-month follow-up, 50% of patients were alive [91].
Given the relatively high risk of relapse after allo-HCT in adverse-risk AML, several maintenance strategies have been implemented to improve outcomes. After encouraging results in early phase studies, a phase III, open-label, randomized trial of AZA maintenance after allo-HCT in high-risk MDS/AML was conducted. The study did not show a survival benefit with AZA maintenance [92]. Most recently, a phase II study was conducted to assess the efficacy and safety of eprenetapopt (small molecule p53 reactivator) combined with AZA as maintenance therapy after allo-HCT in patients with TP53-mutated MDS/AML [93]. Patients received up to 12 cycles of eprenetapopt: 3.7 g once daily intravenously on days 1 through 4 and AZA 36 mg/m2 once daily intravenously or subcutaneously on days 1 through 5 in 4-week cycles. The primary outcomes were safety and relapse-free survival. Thirty-three patients were treated (14 AML, 19 MDS). With a median follow-up of 14.5 months, the median relapse-free survival was 12.5 months (95% CI, 9.6-not reached), and with a median follow-up of 17.0 months, the median OS was 20.6 months (95% CI, 14.2-not reached). The combination had an acceptable toxicity profile, and acute and chronic (all grade) graft-versus-host were reported in 4 (12%) and 11 (33%) patients, respectively. The Phase 3 trial of eprenetapopt (APR-246) with azacitidine for TP53-mutated MDS and AML did not meet its primary endpoint of improved complete response rates, achieving 33.3% CR in the treatment group versus 22.4% in the control group (p = 0.13) [94]. Factors influencing this outcome include the biological heterogeneity of TP53 mutations, which can lead to variable responses across a diverse patient population.
Additionally, using CR as the primary endpoint may have limited the assessment of the drug’s efficacy, as other measures, like OS, could provide a more comprehensive evaluation. While substantial, the trial’s sample size may not have had enough statistical power to detect meaningful differences. Lastly, patients with advanced disease and poorer prognoses may inherently resist treatment, impacting response rates [95]. To improve future trial designs, selecting more relevant endpoints like overall survival and progression-free survival is crucial. Stratifying patients based on specific mutation types can help identify those most likely to benefit from treatment. Longer follow-up periods should be considered to capture delayed responses, and the incorporation of biomarkers could enhance patient selection and provide insights into resistance mechanisms. Addressing these factors may improve the chances of success in future studies for TP53-mutated MDS and AML patients.
We conducted a real-world study under the COMMAND consortium to examine predictors of outcomes with allo-HCT in 370 patients with TP53-mutated AML [9]. Median event-free survival was 12.4 months (95% CI, 6.24-18.55), and the median OS was 24.5 months (95% CI, 21.80-27.25). Conditioning intensity or HMA maintenance did not impact outcomes. Complete remission at day 100 after allo-HCT and the occurrence of chronic graft-versus-host suggest a favorable impact on event-free survival and OS in multivariable analysis [9].
A systematic review and meta-analysis were conducted to evaluate the outcome of allo-HCT in TP53-mutated AML [96]. The review suggested the favorable impact of allo-HCT in improving OS compared to nonallo–HCT strategies. While allo-HCT should not be denied for eligible patients with TP53 mutation, improved pre- and post-transplant strategies are warranted for a better long-term outcome.
Conclusion
TP53-mutated AML presents a unique challenge in management due to its association with complex karyotypes and resistance to conventional and novel therapies. While we have recognized TP53-associated MN as a distinct entity and that the disease should be managed differently, there is no consensus on how to manage these patients. A substantial effort was put forward to develop novel therapies targeting CD47/SIRPα and dysfunctional p53 to address the critical gap; however, these novel agents did not prove effective in randomized trials. Allo-HCT can offer long-term remission in a subset of patients with optimal responses going into transplant. Research efforts need to continue to develop effective therapies to manage this poor prognostic disease. Furthermore, analysis using large databases is warranted to identify predictors of favorable outcomes with allo-HCT.
References
Kandoth C, McLellan MD, Vandin F, Ye K, Niu B, Lu C, et al. Mutational landscape and significance across 12 major cancer types. Nature. 2013;502:333–9.
Joerger AC, Fersht AR. The tumor suppressor p53: from structures to drug discovery. Cold Spring Harb Perspect Biol. 2010;2:a000919.
Stengel A, Kern W, Haferlach T, Meggendorfer M, Fasan A, Haferlach C. The impact of TP53 mutations and TP53 deletions on survival varies between AML, ALL, MDS and CLL: an analysis of 3307 cases. Leukemia. 2017;31:705–11.
Hiwase D, Hahn C, Tran ENH, Chhetri R, Baranwal A, Al-Kali A, et al. TP53 mutation in therapy-related myeloid neoplasm defines a distinct molecular subtype. Blood. 2023;141:1087–91.
Haferlach T. Advancing leukemia diagnostics: Role of Next Generation Sequencing (NGS) in acute myeloid leukemia. Hematol Rep. 2020;12:8957.
Döhner H, Wei AH, Appelbaum FR, Craddock C, DiNardo CD, Dombret H, et al. Diagnosis and management of AML in adults: 2022 recommendations from an international expert panel on behalf of the ELN. Blood. 2022;140:1345–77.
Arber DA, Orazi A, Hasserjian RP, Borowitz MJ, Calvo KR, Kvasnicka H-M, et al. International Consensus Classification of Myeloid Neoplasms and Acute Leukemias: integrating morphologic, clinical, and genomic data. Blood. 2022;140:1200–28.
Badar T, Atallah E, Shallis RM, Goldberg AD, Patel A, Abaza Y, et al. Outcomes of TP53-mutated AML with evolving frontline therapies: Impact of allogeneic stem cell transplantation on survival. Am J Hematol. 2022;97:E232–e5.
Badar T, Atallah E, Shallis R, Saliba AN, Patel A, Bewersdorf JP, et al. Survival of TP53-mutated acute myeloid leukemia patients receiving allogeneic stem cell transplantation after first induction or salvage therapy: results from the Consortium on Myeloid Malignancies and Neoplastic Diseases (COMMAND). Leukemia. 2023;37:799–806.
Bode AM, Dong ZG. Post-translational modification of p53 in tumorigenesis. Nat Rev Cancer. 2004;4:793–805.
Lacroix M, Riscal R, Arena G, Linares LK, Le Cam L. Metabolic functions of the tumor suppressor p53: Implications in normal physiology, metabolic disorders, and cancer. Mol Metab. 2020;33:2–22.
Bieging KT, Mello SS, Attardi LD. Unravelling mechanisms of p53-mediated tumour suppression. Nat Rev Cancer. 2014;14:359–70.
Soenen V, Preudhomme C, Roumier C, Daudignon A, Laï JL, Fenaux P. 17p deletion in acute myeloid leukemia and myelodysplastic syndrome.: Analysis of breakpoints and deleted segments by fluorescence in situ. Blood. 1998;91:1008–15.
Hofstetter G, Berger A, Berger R, Zoric A, Braicu EI, Reimer D, et al. The N-Terminally Truncated p53 Isoform Δ40p53 Influences Prognosis in Mucinous Ovarian Cancer. Int J Gynecol Cancer. 2012;22:372–9.
Brosh R, Rotter V. When mutants gain new powers: news from the mutant p53 field. Nat Rev Cancer. 2009;9:701–13.
Toledo F, Wahl GM. Regulating the p53 pathway: in vitro hypotheses, in vivo veritas. Nat Rev Cancer. 2006;6:909–23.
Bernard E, Nannya Y, Hasserjian RP, Devlin SM, Tuechler H, Medina-Martinez JS, et al. Implications of TP53 allelic state for genome stability, clinical presentation and outcomes in myelodysplastic syndromes. Nat Med. 2020;26:1549–56.
Badar T, Nanaa A, Atallah E, Shallis RM, Craver EC, Li Z, et al. Prognostic impact of ‘multi-hit’ versus ‘single hit’ TP53 alteration in patients with acute myeloid leukemia: results from the Consortium on Myeloid Malignancies and Neoplastic Diseases. Haematologica. 2024;109:3533–42.
Grob T, Al Hinai ASA, Sanders MA, Kavelaars FG, Rijken M, Gradowska PL, et al. Molecular characterization of mutant TP53 acute myeloid leukemia and high-risk myelodysplastic syndrome. Blood. 2022;139:2347–54.
Khoury JD, Solary E, Abla O, Akkari Y, Alaggio R, Apperley JF, et al. The 5th edition of the World Health Organization Classification of Haematolymphoid Tumours: Myeloid and Histiocytic/Dendritic Neoplasms. Leukemia. 2022;36:1703–19.
Swerdlow SH, Campo E, Harris NL, Jaffe ES, Pileri SA, Stein H, et al. WHO classification of tumours of haematopoietic and lymphoid tissues: International agency for research on cancer Lyon, France; 2008.
Estey E, Hasserjian RP, Döhner H. Distinguishing AML from MDS: a fixed blast percentage may no longer be optimal. Blood, J Am Soc Hematol. 2022;139:323–32.
Christiansen DH, Andersen MK, Pedersen-Bjergaard J. Mutations with loss of heterozygosity of p53 are common in therapy-related myelodysplasia and acute myeloid leukemia after exposure to alkylating agents and significantly associated with deletion or loss of 5q, a complex karyotype, and a poor prognosis. J Clin Oncol. 2001;19:1405–13.
Ok CY, Patel KP, Garcia-Manero G, Routbort MJ, Peng J, Tang G, et al. TP53 mutation characteristics in therapy-related myelodysplastic syndromes and acute myeloid leukemia is similar to de novo diseases. J Hematol Oncol. 2015;8:45.
Wong TN, Ramsingh G, Young AL, Miller CA, Touma W, Welch JS, et al. Role of TP53 mutations in the origin and evolution of therapy-related acute myeloid leukaemia. Nature. 2015;518:552–5.
Attardi LD. The role of p53-mediated apoptosis as a crucial anti-tumor response to genomic instability: lessons from mouse models. Mutat Res-Fund Mol M. 2005;569:145–57.
Rücker FG, Schlenk RF, Bullinger L, Kayser S, Teleanu V, Kett H, et al. TP53 alterations in acute myeloid leukemia with complex karyotype correlate with specific copy number alterations, monosomal karyotype, and dismal outcome. Blood, J Am Soc Hematol. 2012;119:2114–21.
Papaemmanuil E, Gerstung M, Bullinger L, Gaidzik VI, Paschka P, Roberts ND, et al. Genomic Classification and Prognosis in Acute Myeloid Leukemia. N. Engl J Med. 2016;374:2209–21.
Shah MV, Tran ENH, Shah S, Chhetri R, Baranwal A, Ladon D, et al. TP53 mutation variant allele frequency of ≥10% is associated with poor prognosis in therapy-related myeloid neoplasms. Blood Cancer J. 2023;13:51.
De Kouchkovsky I, Abdul-Hay M. Acute myeloid leukemia: a comprehensive review and 2016 update. Blood Cancer J. 2016;6, e441.
Moreno Vanegas Y, Badar T. Clinical Utility of Azacitidine in the Management of Acute Myeloid Leukemia: Update on Patient Selection and Reported Outcomes. Cancer Manag Res. 2022;14:3527–38.
Prochazka KT, Pregartner G, Rücker FG, Heitzer E, Pabst G, Wölfler A, et al. Clinical implications of subclonal TP53 mutations in acute myeloid leukemia. Haematologica. 2019;104:516–23.
Rucker FG, Schlenk RF, Bullinger L, Kayser S, Teleanu V, Kett H, et al. Alterations in Acute Myeloid Leukemia with Complex Karyotype Correlate with Specific Copy Number Alterations, Monosomal Karyotype, and Dismal Outcome. Blood. 2011;118:1518.
Yanada M, Yamamoto Y, Iba S, Okamoto A, Inaguma Y, Tokuda M, et al. mutations in older adults with acute myeloid leukemia. Int J Hematol. 2016;103:429–35.
Daver NG, Iqbal S, Renard C, Chan RJ, Hasegawa K, Hu H, et al. Treatment outcomes for newly diagnosed, treatment-naïve TP53-mutated acute myeloid leukemia: a systematic review and meta-analysis. J Hematol Oncol. 2023;16:19.
Lancet JE, Uy GL, Cortes JE, Newell LF, Lin TL, Ritchie EK, et al. CPX-351 (cytarabine and daunorubicin) Liposome for Injection Versus Conventional Cytarabine Plus Daunorubicin in Older Patients With Newly Diagnosed Secondary Acute Myeloid Leukemia. J Clin Oncol. 2018;36:2684–92.
Goldberg AD, Talati C, Desai P, Famulare C, Devlin SM, Farnoud N, et al. TP53 Mutations Predict Poorer Responses to CPX-351 in Acute Myeloid Leukemia. Blood. 2018;132:1433.
Murthy V, Whitmill R, Lodwick C, Dyer P, Ahmed M, Khan R, et al. CPX-351 for Acute Myeloid Leukaemia: Real World Results Are Comparable to Trial Outcomes and Exceeds Them in Non-Adverse Risk Patients-a Multicentre Experience from West Midlands Hospitals on Behalf of West Midlands Research Consortium (WMRC) UK. Blood. 2021;138:4416.
Przespolewski AC, Talati C, Fazal S, Vachhani P, Sanikommu S, Thota S, et al. Safety and efficacy of CPX-351 in younger patients< 60 years old with secondary acute myeloid leukemia: An updated analysis. Am Soc Clin Oncol. 2019.
Lee D, Jain AG, Deutsch Y, Eatrides J, Chan O, Padron E, et al. CPX-351 Yields Similar Response and Survival Outcome in Younger and Older Patients With Secondary Acute Myeloid Leukemia. Clin Lymphoma Myeloma Leuk. 2022;22:774–9.
Grenet J, Jain AG, Burkart M, Waksal J, Famulare C, Numan Y, et al. Comparing outcomes between liposomal daunorubicin/cytarabine (cpx-351) and hma+ venetoclax as frontline therapy in acute myeloid leukemia. Blood. 2021;138:32.
Chiche E, Rahmé R, Bertoli S, Dumas P-Y, Micol J-B, Hicheri Y, et al. Real-life experience with CPX-351 and impact on the outcome of high-risk AML patients: a multicentric French cohort. Blood Adv. 2021;5:176–84.
Ghorab A, Al-Kali A, Elliot M, Gangat N, Alkhateeb H, Shah M, et al. Clinical outcome of myelodysplastic syndrome progressing on hypomethylating agents with evolving frontline therapies: continued challenges and unmet needs. Blood Cancer J. 2022;12:93.
Fenaux P, Mufti GJ, Hellström-Lindberg E, Santini V, Gattermann N, Germing U, et al. Azacitidine Prolongs Overall Survival Compared With Conventional Care Regimens in Elderly Patients With Low Bone Marrow Blast Count Acute Myeloid Leukemia. J Clin Oncol. 2010;28:562–9.
Nieto M, Samper E, Fraga MF, de Buitrago GG, Esteller M, Serrano M. The absence of p53 is critical for the induction of apoptosis by 5-aza-2′-deoxycytidine. Oncogene. 2004;23:735–43.
Welch JS, Petti AA, Miller CA, Fronick CC, O’Laughlin M, Fulton RS, et al. TP53 and Decitabine in Acute Myeloid Leukemia and Myelodysplastic Syndromes. N. Engl J Med. 2016;375:2023–36.
Short NJ, Kantarjian HM, Loghavi S, Huang XL, Qiao W, Borthakur G, et al. Five-Day Versus Ten-Day Schedules of Decitabine in Older Patients with Newly Diagnosed Acute Myeloid Leukemia: Results of a Randomized Phase II Study. Blood. 2018;132.
Hunter AM, Sallman DA. Current status and new treatment approaches in TP53 mutated AML. Best Pr Res Clin Haematol. 2019;32:134–44.
DiNardo CD, Jonas BA, Pullarkat V, Thirman MJ, Garcia JS, Wei AH, et al. Azacitidine and Venetoclax in Previously Untreated Acute Myeloid Leukemia. N. Engl J Med. 2020;383:617–29.
Kim K, Maiti A, Loghavi S, Pourebrahim R, Kadia TM, Rausch CR, et al. Outcomes of TP53-mutant acute myeloid leukemia with decitabine and venetoclax. Cancer. 2021;127:3772–81.
Badar T, Nanaa A, Atallah E, Shallis RM, Guilherme SCC, Goldberg AD, et al. Comparing venetoclax in combination with hypomethylating agents to hypomethylating agent-based therapies for treatment naive TP53-mutated acute myeloid leukemia: results from the Consortium on Myeloid Malignancies and Neoplastic Diseases (COMMAND). Blood Cancer J. 2024;14:32.
DiNardo CD, Tiong IS, Quaglieri A, MacRaild S, Loghavi S, Brown FC, et al. Molecular patterns of response and treatment failure after frontline venetoclax combinations in older patients with AML. Blood. 2020;135:791–803.
DiNardo CD, Pratz K, Pullarkat V, Jonas BA, Arellano M, Becker PS, et al. Venetoclax combined with decitabine or azacitidine in treatment-naive, elderly patients with acute myeloid leukemia. Blood. 2019;133:7–17.
Gangat N, Johnson I, McCullough K, Farrukh F, Al-Kali A, Alkhateeb H, et al. Molecular predictors of response to venetoclax plus hypomethylating agent in treatment-naïve acute myeloid leukemia. Haematologica. 2022;107:2501–5.
Pollyea DA, Pratz KW, Wei AH, Pullarkat V, Jonas BA, Recher C, et al. Outcomes in Patients with Poor-Risk Cytogenetics with or without TP53 Mutations Treated with Venetoclax and Azacitidine. Clin Cancer Res. 2022;28:5272–9.
Gangat N, Karrar O, Iftikhar M, McCullough K, Johnson IM, Abdelmagid M, et al. Venetoclax and hypomethylating agent combination therapy in newly diagnosed acute myeloid leukemia: Genotype signatures for response and survival among 301 consecutive patients. Am J Hematol.
Daver N, Alotaibi AS, Bucklein V, Subklewe M. T-cell-based immunotherapy of acute myeloid leukemia: current concepts and future developments. Leukemia. 2021;35:1843–63.
Yang H, Bueso-Ramos C, DiNardo C, Estecio MR, Davanlou M, Geng QR, et al. Expression of PD-L1, PD-L2, PD-1 and CTLA4 in myelodysplastic syndromes is enhanced by treatment with hypomethylating agents. Leukemia. 2014;28:1280–8.
Ørskov AD, Treppendahl MB, Skovbo A, Holm MS, Friis LS, Hokland M, et al. Hypomethylation and up-regulation of PD-1 in T cells by azacytidine in MDS/AML patients: A rationale for combined targeting of PD-1 and DNA methylation. Oncotarget. 2015;6:9612–26.
Sallman DA, Amy M, Komrokji RS, McGraw K, Geyer SM, Eksioglu E, et al. Immune Checkpoint Profiling of TP53 Mutant and Wild-Type Myeloid Malignancies: TP53 Mutations Direct Immune Tolerance Via an Immunosuppressive Phenotype. Blood. 2017;130:423.
Daver N, Garcia-Manero G, Basu S, Boddu PC, Alfayez M, Cortes JE, et al. Efficacy, Safety, and Biomarkers of Response to Azacitidine and Nivolumab in Relapsed/Refractory Acute Myeloid Leukemia: A Nonrandomized, Open-Label, Phase II Study. Cancer Discov. 2019;9:370–83.
Zeidner JF, Vincent BG, Ivanova A, Moore D, McKinnon KP, Wilkinson AD, et al. Phase II Trial of Pembrolizumab after High-Dose Cytarabine in Relapsed/Refractory Acute Myeloid Leukemia. Blood Cancer Discov. 2021;2:616–29.
Ravandi F, Assi R, Daver N, Benton CB, Kadia T, Thompson PA, et al. Idarubicin, cytarabine, and nivolumab in patients with newly diagnosed acute myeloid leukaemia or high-risk myelodysplastic syndrome: a single-arm, phase 2 study. Lancet Haematol. 2019;6:e480–e8.
Acharya N, Sabatos-Peyton C, Anderson AC. Tim-3 finds its place in the cancer immunotherapy landscape. Journal for immunotherapy of cancer. 2020;8.
Brunner AM, Esteve J, Porkka K, Knapper S, Traer E, Scholl S, et al. Phase Ib study of sabatolimab (MBG453), a novel immunotherapy targeting TIM-3 antibody, in combination with decitabine or azacitidine in high- or very high-risk myelodysplastic syndromes. Am J Hematol. 2024;99:E32–e6.
Zeidan AM, Westermann J, Kovacsovics T, Assouline S, Schuh AC, Kim H-J, et al. AML-484 First Results of a Phase II Study (STIMULUS-AML1) Investigating Sabatolimab + Azacitidine + Venetoclax in Patients With Newly Diagnosed Acute Myeloid Leukemia (ND AML). Clin Lymphoma Myeloma Leuk. 2022;22:S255.
Chao MP, Jaiswal S, Weissman-Tsukamoto R, Alizadeh AA, Gentles AJ, Volkmer J, et al. Calreticulin is the dominant pro-phagocytic signal on multiple human cancers and is counterbalanced by CD47. Sci Transl Med. 2010;2:63ra94–63ra94.
Daver NG, Vyas P, Kambhampati S, Al Malki MM, Larson RA, Asch AS, et al. Tolerability and Efficacy of the Anticluster of Differentiation 47 Antibody Magrolimab Combined With Azacitidine in Patients With Previously Untreated AML: Phase Ib Results. J Clin Oncol. 2023;41:4893–904.
Daver N, Senapati J, Maiti A, Loghavi S, Kadia TM, DiNardo CD, et al. Phase I/II Study of Azacitidine (AZA) with Venetoclax (VEN) and Magrolimab (Magro) in Patients (pts) with Newly Diagnosed (ND) Older/Unfit or High-Risk Acute Myeloid Leukemia (AML) and Relapsed/Refractory (R/R) AML. Blood. 2022;140:141–4.
Gilead Statement on the Discontinuation of Magrolimab Study in AML with TP53 Mutations [press release]. September 26, 2023.
A Study of Evorpacept (ALX148) With Azacitidine for Higher Risk Myelodysplastic Syndrome (ASPEN-02) [Available from: https://clinicaltrials.gov/study/NCT04417517.
A Study of Evorpacept (ALX148) With Venetoclax and Azacitidine for Acute Myeloid Leukemia (ASPEN-05) [Available from: https://clinicaltrials.gov/study/NCT04755244.
Vadakekolathu J, Lai C, Reeder S, Church SE, Hood T, Lourdusamy A, et al. TP53 abnormalities correlate with immune infiltration and associate with response to flotetuzumab immunotherapy in AML. Blood Adv. 2020;4:5011–24.
Watts J, Maris M, Lin TL, Patel P, Madanat YF, Cogle CR, et al. Updated Results from a Phase 1 Study of APVO436, a Novel Bispecific Anti-CD123 x Anti-CD3 Adaptir™ Molecule, in Relapsed/Refractory Acute Myeloid Leukemia and Myelodysplastic Syndrome. Blood. 2022;140:6204–5.
Badar T, Manna A, Gadd ME, Kharfan-Dabaja MA, Qin H. Prospect of CAR T-cell therapy in acute myeloid leukemia. Expert Opin Investig Drugs. 2022;31:211–20.
Zhang H, Bu C, Peng Z, Li G, Zhou Z, Ding W, et al. Characteristics of anti-CLL1 based CAR-T therapy for children with relapsed or refractory acute myeloid leukemia: the multi-center efficacy and safety interim analysis. Leukemia. 2022;36:2596–604.
Sauer T, Parikh K, Sharma S, Omer B, Sedloev D, Chen Q, et al. CD70-specific CAR T cells have potent activity against acute myeloid leukemia without HSC toxicity. Blood. 2021;138:318–30.
Duong VH, Ruppert AS, Mims AS, Borate U, Stein EM, Baer MR, et al. Entospletinib with decitabine in acute myeloid leukemia with mutant TP53 or complex karyotype: A phase 2 substudy of the Beat AML Master Trial. Cancer. 2023;129:2308–20.
ClinicalTrials.gov. Entrectinib in Combination With ASTX727 for the Treatment of Relapsed/Refractory TP53 Mutated Acute Myeloid Leukemia [March 13, 2024]. Available from: https://classic.clinicaltrials.gov/ct2/show/study/NCT05396859.
Yan W, Zhang Y, Zhang J, Liu S, Cho SJ, Chen X. Mutant p53 protein is targeted by arsenic for degradation and plays a role in arsenic-mediated growth suppression. J Biol Chem. 2011;286:17478–86.
Decitabine,Cytarabine and Arsenic Trioxide for Acute Myeloid Leukemia With p53 Mutations [Available from: https://classic.clinicaltrials.gov/ct2/show/NCT03381781].
Parrales A, Ranjan A, Iyer SV, Padhye S, Weir SJ, Roy A, et al. DNAJA1 controls the fate of misfolded mutant p53 through the mevalonate pathway. Nat Cell Biol. 2016;18:1233–43.
Skuli S, Bakayoko AI, Wertheim G, Riley O, Kruidenier M, Manning B, et al. The Mevalonate Pathway Is a Therapeutic Target in TP53 Mutant Acute Myeloid Leukemia. Blood. 2023;142:408.
Nawas MT, Kosuri S. Utility or futility? A contemporary approach to allogeneic hematopoietic cell transplantation for TP53-mutated MDS/AML. Blood Adv. 2024;8:553–61.
Ciurea SO, Chilkulwar A, Saliba RM, Chen J, Rondon G, Patel KP, et al. Prognostic factors influencing survival after allogeneic transplantation for AML/MDS patients with TP53 mutations. Blood. 2018;131:2989–92.
Loke J, Labopin M, Craddock C, Cornelissen JJ, Labussière-Wallet H, Wagner-Drouet EM, et al. Additional cytogenetic features determine outcome in patients allografted for TP53 mutant acute myeloid leukemia. Cancer. 2022;128:2922–31.
Badar T, Litzow MR, Shallis RM, Bewersdorf JP, Saliba A, Stahl M, et al. Multicenter Analysis of Treatment and Outcomes for Patient with TP53 Mutated AML in the Era of Novel Therapies; Significant Impact of Allogeneic Stem Cell Transplantation on Survival. Blood. 2021;138:797.
Short NJ, Montalban-Bravo G, Hwang H, Ning J, Franquiz MJ, Kanagal-Shamanna R, et al. Prognostic and therapeutic impacts of mutant TP53 variant allelic frequency in newly diagnosed acute myeloid leukemia. Blood Adv. 2020;4:5681–9.
Shirole NH, Pal D, Kastenhuber ER, Senturk S, Boroda J, Pisterzi P, et al. TP53 exon-6 truncating mutations produce separation of function isoforms with pro-tumorigenic functions. Elife. 2016;5.
Lindsley RC, Saber W, Mar BG, Redd R, Wang T, Haagenson MD, et al. Prognostic Mutations in Myelodysplastic Syndrome after Stem-Cell Transplantation. N. Engl J Med. 2017;376:536–47.
Garcia JS, Kim HT, Murdock HM, Cutler CS, Brock J, Gooptu M, et al. Adding venetoclax to fludarabine/busulfan RIC transplant for high-risk MDS and AML is feasible, safe, and active. Blood Adv. 2021;5:5536–45.
Oran B, de Lima M, Garcia-Manero G, Thall PF, Lin R, Popat U, et al. A phase 3 randomized study of 5-azacitidine maintenance vs observation after transplant in high-risk AML and MDS patients. Blood Adv. 2020;4:5580–8.
Mishra A, Tamari R, DeZern AE, Byrne MT, Gooptu M, Chen YB, et al. Eprenetapopt Plus Azacitidine After Allogeneic Hematopoietic Stem-Cell Transplantation for TP53-Mutant Acute Myeloid Leukemia and Myelodysplastic Syndromes. J Clin Oncol. 2022;40:3985–93.
Aprea Therapeutics announces results of primary endpoint from phase 3 trial of eprenetapopt in TP53 mutant myelodysplastic syndromes (MDS) [press release]. Published Dec 28, 2020.
Tucker N. Minimal Efficacy Observed with Eprenetapopt Plus Azacitidine in TP53-Mutant MDS. Targeted Oncol.
Shahzad M, Tariq E, Chaudhary SG, Anwar I, Iqbal Q, Fatima H, et al. Outcomes with allogeneic hematopoietic stem cell transplantation in TP53-mutated acute myeloid leukemia: a systematic review and meta-analysis. Leukemia Lymphoma. 2022:1-9.
Bally C, Adès L, Renneville A, Sebert M, Eclache V, Preudhomme C, et al. Prognostic value of TP53 gene mutations in myelodysplastic syndromes and acute myeloid leukemia treated with azacitidine. Leuk Res. 2014;38:751–5.
Uy GL, Aldoss I, Foster MC, Sayre PH, Wieduwilt MJ, Advani AS, et al. Flotetuzumab as salvage immunotherapy for refractory acute myeloid leukemia. Blood J Am Soc Hematol. 2021;137:751–62.
Cluzeau T, Sebert M, Rahmé R, Cuzzubbo S, Lehmann-Che J, Madelaine I, et al. Eprenetapopt Plus Azacitidine in TP53-Mutated Myelodysplastic Syndromes and Acute Myeloid Leukemia: A Phase II Study by the Groupe Francophone des Myélodysplasies (GFM). J Clin Oncol. 2021;39:1575–83.
Acknowledgements
The Scientific Publications staff at Mayo Clinic provided copyediting, proofreading, administrative, and clerical support.
Author information
Authors and Affiliations
Contributions
MS: Conceptualization and writing original draft. MKA, NGD, MVS, DH, DA and MKD: Review, and editing the manuscript. TB: Conceptualization, supervision, review, and editing the manuscript.
Corresponding author
Ethics declarations
Competing interests
TB: Served on the advisory board for Pfizer, Morphosys, and Takeda.
Additional information
Publisher’s note Springer Nature remains neutral with regard to jurisdictional claims in published maps and institutional affiliations.
Rights and permissions
Open Access This article is licensed under a Creative Commons Attribution-NonCommercial-NoDerivatives 4.0 International License, which permits any non-commercial use, sharing, distribution and reproduction in any medium or format, as long as you give appropriate credit to the original author(s) and the source, provide a link to the Creative Commons licence, and indicate if you modified the licensed material. You do not have permission under this licence to share adapted material derived from this article or parts of it. The images or other third party material in this article are included in the article’s Creative Commons licence, unless indicated otherwise in a credit line to the material. If material is not included in the article’s Creative Commons licence and your intended use is not permitted by statutory regulation or exceeds the permitted use, you will need to obtain permission directly from the copyright holder. To view a copy of this licence, visit http://creativecommons.org/licenses/by-nc-nd/4.0/.
About this article
Cite this article
Shahzad, M., Amin, M.K., Daver, N.G. et al. What have we learned about TP53-mutated acute myeloid leukemia?. Blood Cancer J. 14, 202 (2024). https://doi.org/10.1038/s41408-024-01186-5
Received:
Revised:
Accepted:
Published:
DOI: https://doi.org/10.1038/s41408-024-01186-5